The spacecraft
Contents
[hide]Overview[edit]
The Planck spacecraft was designed, built and tested around two major modules.
- The payload module containing: an off-axis telescope with a projected diameter of 1.5m, focussing radiation from the sky onto a focal plane shared by detectors of the LFI and HFI, operating at 20K and 0.1K, respectively; a telescope baffle that simultaneously provides stray-light shielding and radiative cooling; and three conical “V-groove” baffles that provide thermal and radiative insulation between the warm service module and the cold telescope and instruments.
- The service module containing all the warm electronics servicing instruments and spacecraft, and the solar panel providing electrical power. It also contains the cryocoolers, the main on-board computer, the telecommand receivers and telemetry transmitters, and the attitude control system with its sensors and actuators.
The figures and Table below show the major elements and characteristics of the Planck satellite.
Diameter | 4.2 m | Defined by the solar array |
Height | 4.2 m | |
Total mass at launch | 1912 kg | Fuel mass = 385 kg at launch; He mass = 7.7 kg |
Electrical power demand (avg) | 1300 W | Instrument part: 685 W (Begining of Life); 780 W (End of Life) |
Minimum operational lifetime | 18 months | Planck operated for 32 months with both instruments; the LFI continued surveying the sky for an additional 20 months |
Spin rate | 1 rpm | ±0.6 arcmin/sec (changes due to manoeuvers); stability during inertial pointing ≈6.5×10-5 rpm/h |
Max angle of spin axis to Sun | 10° | To maintain the payload in the shade; default angle is 7.5° |
Max angle of spin axis to Earth | 15° | To allow communication with Earth |
Angle between spin axis and telescope boresight | 85° | Max extent of FOV ≈8° |
On-board data storage capacity | 32 Gbit | Two redundant units (only one is operational at any time) |
Data transmission rate to ground (max) | 1.5 Mbps | Within 15° of Earth, using a 35-m ground antenna |
Daily contact period | Between 2 and 4 hours (as required by operational needs) | Effective real-time science data acquisition bandwidth 130 kbps |
A slightly more detailed overview of the satellite can be found in Planck-PreLaunch-I[1].
A more complete technical description of the Planck satellite can be found in Satellite_UM_1[2] and Satellite_UM_2[3].
The payload module[edit]
The following figure sketches out the main elements of the payload module.
The telescope[edit]
The telescope is an off-axis aplanatic design with two elliptical reflectors, a 1.5-m projected diameter, and an overall emissivity below about 1\%. The optical system was optimized for a set of representative detectors (eight HFI and eight LFI).
The Planck telescope assembly consists of:
- the primary and secondary reflectors (PR and SR), designed and manufactured by Astrium (Friedrichshafen, Germany);
- the support structure, designed and manufactured by Oerlikon Space (Zürich, Switzerland).
- the baffle surrounding the telescope, designed and manufactured by Contraves (Zürich, Switzerland)
- the third V-groove which forms the floor of the cavity in which the telescope and focal plane are located, designed and manufactured by Thales Alenia Space (Cannes, France).
The telescope focusses radiation from the sky onto the focal plane, which consists of two arrays of detector feedhorns, designed, manufactured and tested by the LFI and HFI instrument teams (Planck-PreLaunch-VI[4], Planck-PreLaunch-VII[5], Planck-PreLaunch-XII[6]).
A more detailed description of the Planck telescope can be found in Planck-PreLaunch-II[7]. The in-flight performance of the telescope is investigated in (XXX).
The instruments[edit]
The frequency range of the two Planck instruments together is designed to cover the peak of the CMB spectrum and to characterize the spectra of the main Galactic foregrounds (synchrotron and free-free emission at low frequencies, and dust emission at high frequencies). The LFI covers 30–70GHz in three bands, and the HFI covers 100–857 GHz in six bands. The band centers are spaced approximately logarithmically. The following figure shows the main elements of the instruments, including the cryogenic focal plane and the warm readout units.
The LFI (Planck-PreLaunch-IV[8]) is designed around 22 pseudo-correlation radiometers fed by corrugated feedhorns and ortho-mode transducers to separate two orthogonal linear polarisations. Each horn thus feeds two radiometers. The radiometers are based on front-end low noise amplifiers using indium-phosphide high electron mobility transistors, which process simultaneously the signal from the sky fed by the telescope, and the signal from stable blackbody reference loads located on the external body of the HFI where they can be maintained at a temperature of 4 K. The front-end amplifiers are located in the focal plane of the telescope, and are operated at a temperature of about 20 K; they feed warm back-end amplifiers and detection electronics via waveguides connecting the FPU to the warm service module. For each radiometer, sky and load time-ordered data are separately reconstructed and made available for processing on the ground.
The HFI Planck-PreLaunch-IX[9] is designed around 52 bolometers fed by corrugated feedhorns and bandpass filters within a back-to-back conical horn optical waveguide. Twenty of the bolometers (spider-web bolometers or SWBs) are sensitive to total power, and the remaining 32 units are arranged in pairs of orthogonally-oriented polarisation-sensitive bolometers (PSBs). All bolometers are operated at a temperature of around 0.1 K, and read out by an AC-bias scheme through JFET amplifiers operated at 130 K.
The main parameters of the instruments at detector level, as derived from early in-flight operations, are summarized in the following table (from Planck-Early-I[10]).
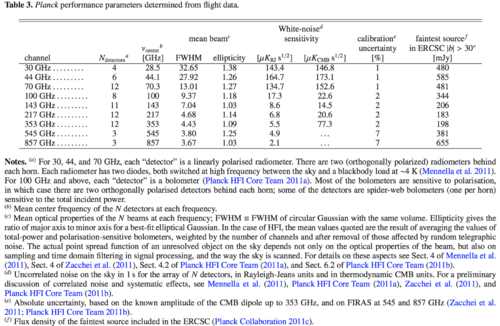
The instrumental performance integrated over all detectors is more appropriately measured at map level. The following tables show the main characteristics of the PR2 (2015) maps.
More information on the LFI and HFI instruments can be found in the following locations.
- A more complete overview of the two Planck instruments can be found here: HFI, LFI.
- A detailed technical description of the instruments can be found in the LFI, HFI, and SCS User Manuals: LFI_UM[12], HFI_UM[13], SCS_UM[14].
- A detailed description of the LFI design and performance as measured on the ground can be found in Planck-PreLaunch-IV[8], Planck-PreLaunch-V[15], and other related papers. Descriptions of the LFI's in-orbit performance can be found in Planck-2020-A2[16], Planck-2015-A02[17] and associated papers, Planck-2013-II[18] and associated papers, and Planck-Early-III[19].
- Detailed descriptions of the HFI design and performance as measured on the ground are included in Planck-PreLaunch-IX[9], Planck-PreLaunch-X[20], and other related papers. Descriptions of the HFI's in-orbit performance can be found in Planck-2020-A3[21], Planck-2015-A07[22] and Planck-2015-A08[23], Planck-2013-VI[24] and associated papers, and Planck-Early-IV[25].
The service module[edit]
The service module provides the various equipment and instruments housed in it with suitable mechanical and thermal environments during launch and orbit. It is formed by an octagonal box, built around a conical tube, which supports the following subsystems intervening in flight operations:
- Command and data management;
- Attitude control and measurement;
- Power control;
- Reaction control;
- Telemetry, tracking and command;
- Thermal control.
The service module contains all the warm satellite and payload electronic units, with the only exception of the box containing JFETs for impedance-matching to the HFI bolometers, which is mounted on the primary reflector support panel, to allow the operation of the JFETs at an optimal temperature of around 130 K. The service module holds:
- Main computer;
- Attitude control computer;
- Data processing units;
- Star trackers;
- LFI electronics;
- Dilution control unit;
- HFI readout electronics;
- 4K compressors;
- Helium tanks, 36000 litres of 4He, and 12000 litres of 3He gas stored in four high-pressure tanks;
- Sorption cooler radiator;
- Sorption cooler electronics;
- Sorption compressors (two redundant units);
- Hydrazine tanks;
- Medium-gain antenna;
- Telemetry subsystem.
More information on each of the subsystems can be found in Satellite_UM_CDMS[26], Satellite_UM_ACMS[27], Satellite_UM_PCS[28], Satellite_UM_RCS[29], Satellite_UM_TTC[30], Satellite_UM_TCS[31].
Thermal design and the cryo-chain[edit]
The cryogenic temperatures required by the detectors are achieved through a combination of passive radiative cooling and three active refrigerators. The contrast between the high power dissipation in the warm service module (1000 W at 300 K) and that at the coldest spot in the satellite (100 nW at 0.1 K) are testimony to the extraordinary efficiency of the complex thermal system which has to achieve such disparate ends simultaneously while preserving a very high level of stability at the cold end. The telescope baffle and V-groove shields are key parts of the passive thermal system. The baffle (which also acts as a stray-light shield) is a high-efficiency radiator consisting of 14 m2 of open aluminium honeycomb coated with black cryo- genic paint; the effective emissivity of this combination is very high (>0.9). The “V-grooves” are a set of three conical shields with an angle of 5° between adjacent shields; the surfaces (approx 10 m2 on each side) are specular (aluminum coating with an emissivity of 0.045) except for the outer (4.5 m2) area of the topmost V-groove which has the same high-emissivity coating as the baffle. This geometry provides highly efficient radiative coupling to cold space, and a high degree of thermal and radia- tive isolation between the warm spacecraft bus and the cold telescope, baffle, and instruments. The cooling provided by the passive system leads to a temperature of 40–45 K for the telescope and baffle. The active refrigeration chain further reduces the detector temperatures to 20 K (LFI front-end low noise amplifiers) and 0.1 K (HFI bolometers) respectively. It is based on three distinct units working in series (see the figure below).
1. The 20-K hydrogen sorption cooler was designed and built expressly for Planck at NASA’s Jet Propulsion Laboratory (USA); it directly cools the LFI low-noise amplifiers to their operating temperature while providing pre-cooling for the HFI cooler chain. The sorption cooler consists of two cold redundant units, each including a six-element sorption compressor and a Joule-Thomson (JT) expansion valve. Each element of the compressor is filled with hydride material (La Ni4.78 Sn0.22) which alternately absorbs and releases hy- drogen gas under control of a heat source. The cooler pro- duces liquid hydrogen in two liquid-vapor heat-exchangers (LVHXs) whose temperatures are stabilized by hydrogen ab- sorption into three compressor elements. LVHX1 provides pre-cooling for the HFI 4-K cooler, while LVHX2 cools the LFI focal plane unit (FPU). The vapor pressure of the liquid hydrogen in the LVHXs is determined primarily by the absorption isotherms of the hydride material used in the compressor elements. Thus, the heat rejection temperature of the compressor elements determines the instrument temperatures. On the spacecraft the compressor rejects heat to a radiator to space with flight allowable temperatures between 262 and 282 K; the radiator is a single unit which couples the active and redundant sorption coolers via a network of heat pipes. The operating efficiency of the Planck sorption cooler depends on passive cooling by radiation to space, which is accomplished by heat exchange of the gas piping to the three V-groove radiators. The final V-groove was required to be between 45 and 60 K to provide the required cooling power for the two instruments. At the expected operating temperature of 47 K, with a working pressure of 3.2 MPa, the two sorption coolers produce the 990 mW of required cooling power for the two instruments, with a margin of approximately 100 mW. The temperature in flight at the heat exchangers were typically 17.5 K (LVHX1) and 19 K (LVHX2). LVHX2 is actively stabilised by a closed loop heat control.
2. The 4-K Joule-Thomson cooler was based on the closed circuit JT expansion of helium, driven by two mechanical compressors, one for the high pressure side and one for the low pressure side. Similar compressors have already been used for active cooling at 70 K in space. The Planck 4-K cooler was initially developed under an ESA programme to provide 4-K cooling with reduced vibration for the Herschel (then named FIRST) observatory. For this reason the two compressors are mounted in a back-to-back configuration, which cancels most of the mo- mentum transfer to the spacecraft. Furthermore, force transducers placed between the two compressors provide an error signal which is used by the drive electronics servo system to control the motion profile of the pistons up to the 7th harmonic of the base compressor frequency (40 Hz). The damping of vibration achieved by this system is more than two orders of magnitude at the base frequency and fac- tors of a few at higher harmonics; the residual vibration levels had no detectable heating effect on the 100 mK stage, and negligible impact on the pointing. Pre-cooling of the helium was provided by the sorption cooler described above. The cold end of the cooler consisted of a liquid helium reservoir located just behind the JT orifice. This cold tip was attached to the bottom of the 4-K box of the HFI FPU. It provided cooling for this screen and also pre-cooling for the gas in the dilution cooler pipe described later in this sec- tion. The margin between heat lift and heat load depended sensitively on the pre-cooling temperature provided by the sorption cooler at the LVHX1 interface. The temperature of LVHX1 was thus the most critical interface of the HFI cryogenic chain; system-level tests showed that it was likely to be around 17.5 K, about 2 K below the maximum requirement. At this pre-cooling temperature the heat load was 10.6 mW and the heat lift was 16.1 mW for a compressor stroke amplitude of 3.5 mm (the maximum is 4.4 mm). The heat load of the 4-K cooler onto the sorption cooler was only 30 mW, a very small amount with respect to the heat lift of the sorption cooler (990 mW); thus there was little back reaction of the 4 K onto the sorption cooler.
3. The dilution cooler consisted of two cooling stages in series, using 36000 litres of 4He and 12000 litres of 3He gas stored on-board in 4 high-pressure tanks. The first stage was based on JT expansion, and produced cooling for the 1.6 K screen of the FPU and for pre-cooling of the second stage cooler. The latter was based on a dilution cooler principle working at zero-G, which was invented and tested by A. Benoît of Institut Neel (Grenoble, F), and developed into a space-qualified system by the Institut d’Astrophysique Spatiale (Orsay) and DTA Air Liquide (Grenoble). The gas from the tanks (at 300 bars at the start of the mission) was brought down to 19 bars through a pressure regulator and the flow through the dilution was regulated by a set of discrete restrictions which could be switched by telecommand. The gas was vented to space after the dilution process, and the cooler therefore had a lifetime limited by the gas supply. The dilution of the two helium isotopes provided the cooling of the bolometers to a temperature around 100 mK which was required to deliver a very high sensitivity for the channels near the peak of the CMB spectrum (noise equivalent power around 10−17 W/√Hz), limited mostly by the background photon noise.
The flight system was at ambient temperature at launch and cooled in space to operating conditions. The HFI bolometer plate reached 93 mK on 3 July 2009, 50 days after launch. The solar panel always faced the Sun, shadowing the rest of Planck, and operated at a mean temperature of 384 K. At the other end of the spacecraft, the telescope baffle operated at 42.3 K and the telescope primary mirror operated at 35.9 K. The temperatures of key parts of the instruments were stabilized by both active and passive methods. Temperature fluctuations were driven by changes in the distance from the Sun, sorption cooler cycling and fluctuations in gas-liquid flow, and fluctuations in cosmic ray flux on the dilution and bolometer plates. These fluctuations did not compromise the science data.
A detailed description of the cooling system and its performance in flight can be found in Planck-Early-II[32].
Pointing[edit]
Planck spun at 1 rpm around the axis of symmetry of the solar panel. In flight, the solar panel could be pointed within a cone of 10° around the direction to the Sun; everything else was always in its shadow. The attitude control relied principally on two systems.
- Redundant star trackers as the main sensors, and solar cells for rough guidance and anomaly detection. The star trackers contained CCDs, which were read out in synchrony with the speed of the field-of-view across the sky to keep star images compact.
- Redundant sets of hydrazine 20-N thrusters for large manoeuvres and 1-N thrusters for fine manoeuvres.
An on-board computer dedicated to this task read out the star trackers at a frequency of 4 Hz, and determined in real time the absolute pointing of the satellite based on a catalogue of bright stars. Manoeuvres were carried out as a sequence of 3 or 4 thrusts spaced in time by integer spin periods, whose duration was calculated on-board, with the objective to achieve the requested attitude with minimal excitation of nutation. There was no further active damping of nutation during periods of inertial pointing, i.e. between manoeuvres. The duration of a small manoeuvre typical of routine operations (2 arcmin) was about 5 min. Larger manoeuvres were achieved by a combination of thrusts using both 1-N and 20-N thrusters, and their duration could be considerable (up to several hours for manoeuvre amplitudes of several degrees). The attitudes measured on-board were further filtered on the ground to reconstruct with high accuracy the spacecraft attitude (or rather the star tracker reference frame). The star trackers and the instrumental field-of-view were aligned on the ground independently to the spacecraft reference frame; the resulting alignment accuracy between the star trackers and the instruments was of 0◦.19, far better than required. The angles between the star tracker frame and each of the detectors were determined in flight from observations of planets. Several bright planets drifted through the field-of-view once every 6 months, providing many calibration points every year. There are many weaker point sources, both celestial and in the Solar System, which provided much more frequent though less accurate calibration tests. The in-flight pointing calibration was very robust vis-a-vis the expected thermoelastic deformations (which were expected to contribute a total of 0.14 arcmin to the total on-ground alignment budget).
The 20-N thrusters were also used for orbit control manoeuvres during transfer to the final Planck orbit (two large manoeuvres planned) and for orbit maintenance (typically one manoeuvre per month). Most of the hydrazine thruster fuel that Planck carried was expended in the two large manoeuvres carried out during transfer, and a very minor amount was required for orbit maintenance.
A more detailed technical description of the pointing subsystem can be found in Satellite_UM_ACMS[27]. A summary assessment of the pointing performance during the first year of operations can be found in Planck-Early-I[10] and in this Explanatory Supplement (under Routine Operations).
Additional instruments[edit]
The Standard Radiation Environment Monitor[edit]
The standard radiation environment monitor (SREM)[33] is a particle detector developed for satellite applications and carried on board many ESA satellites, including Planck. It measures high-energy electrons and protons of the space environment with ±20° angular resolution, as well as spectral information, and provides the host spacecraft with radiation data. SREM was developed and manufactured by Contraves Space in cooperation with the Paul Scherrer Institute under a development contract of the European Space Agency. SREM is the second generation of instruments in a programme that was established by ESA’s European Research and Technology Centre (ESTEC) to:
- provide minimum intrusive radiation detectors for space applications;
- provide radiation hazard alarm function to instruments on board spacecraft;
- assist in investigation activities related to possible radiation-related anomalies observed on spacecraft;
- assist in in-flight Technology Demonstration Activities.
The design goals are low weight, small dimensions, and low power consumption, combined with the ability to provide particle species and spectral information.
In the case of Planck, the use of the SREM was entirely within the scope of bullets 1 and 3 above, since, due to the nature of operations, no real time alarms were possible.
The SREM consists of three detectors (D1, D2, and D3) in two detector head configurations. One system is a single silicon diode detector (D3). The main entrance window is covered with 0.7 mm aluminum, which defines the lower energy threshold for electrons to about 0.5 MeV and for protons to about 10MeV. The other system uses two silicon diodes (detectors D1/D2) arranged in a telescope configuration. The main entrance of this detector is covered with 2 mm of aluminium giving a proton and electron threshold of 20 and 1.5 MeV, respectively. A 1.7-mm-thick aluminium and 0.7–mm-thick tantalum layer separate the two diodes of the telescope configuration.
The telescope detector allows measurement of the high-energy proton fluxes with enhanced energy resolution. In addition, the shielding between the two diodes in the telescope prevents the passage of electrons. However, protons with energies greater than about 30 MeV go through. Thus, using the two diodes in coincidence gives pure proton count rates, allowing subtraction of the proton contribution from the electron channels. A total of 15 discriminator levels (see table below) are available to bin the energy of the detected events.
Name | Logic | Particle | Energy [MeV] |
---|---|---|---|
TC1 | D1 | protons | >20 |
S12 | D1 | protons | 20 - 550 |
S13 | D1 | protons | 20 - 120 |
S14 | D1 | protons | 20 - 27 |
S15 | D1 | protons | 20 - 34 |
TC2 | D2 | protons | >39 |
S25 | D2 | ions | 150 - 185 |
C1 | D1 | D2coinc. protons | 40 - 50 |
C2 | D1 | D2coinc. protons | 50 - 70 |
C3 | D1 | D2coinc. protons | 70 - 120 |
C4 | D1 | D2coinc. protons | >130 |
TC3 | D3 | electrons | >0.5 |
S32 | D3 | electrons | 0.55 - 2.3 |
S33 | D3 | protons | 11 - 90 |
S34 | D3 | protons | 11 - 30 |
PL1 | D1 | Dead Time | NA |
PL2 | D2 | Dead Time | NA |
PL3 | D3 | Dead Time | NA |
The detector electronics are capable of processing a detection rate of 100 kHz with dead-time correction below 20%. The SREM is contained in a single box of size 20cm × 12cm × 10cm and weighs 2.6 kg. The box contains the detector systems with the analogue and digital front-end electronics, a power supply, and a TTC-B-01 telemetry and Telecommand interface protocol. By virtue of a modular buildup, the interface can be adapted to any spacecraft system. The power consumption is approximately 2.5 W.
Particle fluxes measured by the SREM on board Planck are shown in the figure below. The radiation environment of Planck was characterised by its operation near the minimum in the solar cycle. As a consequence, the particle flux is dominated by Galactic cosmic rays, rather than by the solar wind. The time evolution of the SREM measurements is well correlated with that of identical units flying simultaneously on other satellites (e.g., Herschel, Rosetta) and with indicators of Galactic cosmic rays, and is anti-correlated with solar flare events and with the solar cycle. More importantly for Planck, the SREM measurements are very well correlated with the heat deposition on the coldest stages of the HFI, and with glitch rates measured by the detectors of HFI. A more detailed interpretation of these data is provided in Planck-Early-IV[25].
The SREM data are provided in the Planck Legacy Archive in the form of individual FITS files per calendar day. The SREM data are described here. Planck SREM data are also available via the Paul Scherrer Institute's SREM page at [[1]].
More technical details on the SREM can be found in {{PlanckPapers|Satellite_UM_5}.
The Fibre-optic gyro unit[edit]
The fibre-optic gyro unit (FOG) was flown on Planck as an experimental unit, and was not used as part of the attitude control system. The FOG principle of operation is based on the physical phenomenon called the Sagnac effect: "a solid-state optical interferometer enclosing a surface located on a rotating support will detect a phase difference of the optical signal, which is proportional to the angular rate and to the surface enclosed by the optical path".
The FOG features the following architecture.
- An optical path enclosing a maximal surface: this is realized through the 1 km long fibre optic, wound many times round, in order to make a reasonable sized interferometer (< 120 mm in diameter).
- A source, to deliver the light to the interferometer.
- A multifunction Integrated Optical Circuit, which closes the interferometer, to share the light between the two extremities of the fibre-optic coil, to filter undesirable polarization.
- A coupler to extract the optical signal coming back from the ring interferometer, which is the signal carrying the Sagnac inertial information, and to direct it towards an optical detector.
- A detection module to convert this light signal into an electrical signal.
- A closed-loop signal processing system to increase the dynamic range and remove the effect of fluctuations of the optical power and of the gain of the detection chain, allowing an easy auto-calibration of the system and excellent scale factor stability and linearity.
- A biasing modulation allowing the measurement of very low rotation rates as well as the sign of those rotations.
Redundancy is offered by providing four skewed gyro channels, allowing the Attitude Control and Measurement Subsystem to use any triplet among the four axes to determine the spacecraft three axis rates. Nominally the four channels are activated, but in case of failure or critical thermal situations a subset of channels could be used.
Although the FOG data was not used in the active control loop of the Planck attitude system, its measurements can be used to improve pointing estimation. A specific set of alternative pointing products which include FOG data were generated by the MOC, but were not used in the processing pipelines of Planck.
More technical details on the FOG can be found in {{PlanckPapers|Satellite_UM_5}.
References[edit]
- Jump up ↑ Planck pre-launch status: The Planck mission, J. A. Tauber, N. Mandolesi, J.-L. Puget, et al. , A&A, 520, A1+, (2010).
- Jump up ↑ 'SATELLITE USERS MANUAL, Chapter 1: Introduction and Mission Definition
- Jump up ↑ 'SATELLITE USERS MANUAL, Chapter 2: System Definition
- Jump up ↑ Planck pre-launch status: Calibration of the Low Frequency Instrument flight model radiometers, F. Villa, L. Terenzi, M. Sandri, et al., A&A, 520, A6+, (2010).
- Jump up ↑ Planck pre-launch status: Low Frequency Instrument optics, M. Sandri, F. Villa, M. Bersanelli, et al. , A&A, 520, A7+, (2010).
- Jump up ↑ Planck pre-launch status: HFI beam expectations from the optical optimisation of the focal plane, B. Maffei, F. Noviello, J. A. Murphy, et al. , A&A, 520, A12+, (2010).
- Jump up ↑ Planck pre-launch status: The optical system, J. A. Tauber, H. U. Nørgaard-Nielsen, P. A. R. Ade, et al. , A&A, 520, A2+, (2010).
- ↑ Jump up to: 8.08.1 Planck pre-launch status: Design and description of the Low Frequency Instrument, M. Bersanelli, N. Mandolesi, R. C. Butler, et al. , A&A, 520, A4+, (2010).
- ↑ Jump up to: 9.09.1 Planck pre-launch status: The HFI instrument, from specification to actual performance, J.-M. Lamarre, J.-L. Puget, P. A. R. Ade, et al. , A&A, 520, A9+, (2010).
- ↑ Jump up to: 10.010.110.2 Planck early results. I. The Planck mission, Planck Collaboration I, A&A, 536, A1, (2011).
- ↑ Jump up to: 11.011.1 Planck 2015 results. I. Overview of products and results, Planck Collaboration, 2016, A&A, 594, A1.
- Jump up ↑ LFI USERS MANUAL, LFI Users Manual
- Jump up ↑ HFI USERS MANUAL, HFI Users Manual
- Jump up ↑ SCS USERS MANUAL, SCS Users Manual
- Jump up ↑ Planck pre-launch status: Low Frequency Instrument calibration and expected scientific performance, A. Mennella, M. Bersanelli, R. C. Butler, et al. , A&A, 520, A5+, (2010).
- Jump up ↑ Planck 2018 results. II. Low Frequency Instrument data processing, Planck Collaboration, 2020, A&A, 641, A2.
- Jump up ↑ Planck 2015 results. II. LFI processing, Planck Collaboration, 2016, A&A, 594, A2.
- Jump up ↑ Planck 2013 results. II. Low Frequency Instrument data processing, Planck Collaboration, 2014, A&A, 571, A2.
- Jump up ↑ Planck early results. III. First assessment of the Low Frequency Instrument in-flight performance, A. Mennella, R. C. Butler, A. Curto, et al. , A&A, 536, A3, (2011).
- Jump up ↑ Planck pre-launch status: HFI ground calibration, F. Pajot, P. A. R. Ade, J.-L. Beney, et al. , A&A, 520, A10+, (2010).
- Jump up ↑ Planck 2018 results. III. High Frequency Instrument data processing and frequency maps, Planck Collaboration, 2020, A&A, 641, A3.
- Jump up ↑ Planck 2015 results. VII. High Frequency Instrument data processing: Time-ordered information and beam processing, Planck Collaboration, 2016, A&A, 594, A7.
- Jump up ↑ Planck 2015 results. VIII. High Frequency Instrument data processing: Calibration and maps, Planck Collaboration, 2016, A&A, 594, A8.
- Jump up ↑ Planck 2013 results. VI. High Frequency Instrument Data Processing, Planck Collaboration, 2014, A&A, 571, A6.
- ↑ Jump up to: 25.025.1 Planck early results, IV. First assessment of the High Frequency Instrument in-flight performance, Planck HFI Core Team, A&A, 536, A4, (2011).
- Jump up ↑ 'SATELLITE USERS MANUAL, Chapter 4: Central Data Management System
- ↑ Jump up to: 27.027.1 'SATELLITE USERS MANUAL, Chapter 4: Attitude Control Management System
- Jump up ↑ 'SATELLITE USERS MANUAL, Chapter 4: Power Control System
- Jump up ↑ 'SATELLITE USERS MANUAL, Chapter 4: Reaction Control System
- Jump up ↑ 'SATELLITE USERS MANUAL, Chapter 4: Telemetry and Telecommand System
- Jump up ↑ 'SATELLITE USERS MANUAL, Chapter 4: Thermal Control System
- Jump up ↑ Planck early results. II. The thermal performance of Planck, Planck Collaboration II, A&A, 536, A2, (2011).
- Jump up ↑ The ESA Standard Radiation Environment Monitor program first results from PROBA-I and INTEGRAL, A. Mohammadzadeh, H. Evans, P. Nieminen, E. Daly, P. Vuilleumier, P. Buhler, C. Eggel, W. Hajdas, N. Schlumpf, A. Zehnder, J. Schneider, R. Fear, Nuclear Science, IEEE Transactions on, 50, 2272-2277, (Dec.).
(Planck) Low Frequency Instrument
(Planck) High Frequency Instrument
revolutions per minute
Field-Of-View
Cosmic Microwave background
Focal Plane Unit
Junction Field Elect Transistor
Sorption Cooler Subsystem (Planck)
European Space Agency
Space Radiation Environment Monitor
European Space TEchnology and Research Centre
Flexible Image Transfer Specification
Fiber Optic Gyroscope
[ESA's] Mission Operation Center [Darmstadt, Germany]